Methods
The original publication is available at www.springerlink.com
Gordon C. K. Roberts
Encyclopedia of Biophysics
10.1007/978-3-642-16712-6_63
© European Biophysical Societies' Association (EBSA) 2013
Flash Photolysis
Igor Chizhov
Institute for Biophysical Chemistry, Hannover Medical School,
Synonyms
Pulsed-laser transient spectroscopy; Time-resolved light-induced reactions
Definition
Flash photolysis is “a technique of transient spectroscopy and transient kinetic studies in which a light pulse is used to produce transient species. Commonly, an intense pulse of short duration is used to produce a sufficient concentration of a transient species suitable for spectroscopic observation” (IUPAC 1997).
Introduction
In 1967 Ronald Norrish, George Porter, and Manfred Eigen were awarded the Nobel Prize for studies of extremely fast chemical reaction, effected by disturbing the equilibrium by means of very short impulses of energy. The flash photolysis method is one those famous relaxation techniques which were developed by laureates and many other scientists in the last half of twentieth century. As it happens quite often in history of science, a technique developed in wartime has been applied in unexpected way. In 1948, George Porter realized that high intensity flash tubes designed for nighttime aerial photography during World War II can generate a concentration of chemical species (free radicals, transient triplet states, etc.) large enough to be observed spectroscopically. Moreover, he decided to use two flash tubes: one powerful 2 ms flash for excitation of reaction and a second weaker but shorter (ca 50 μs) flash of light which passed the reaction volume after particular delay time. This light then hits detector (a spectrograph with photographic plate) and allowed the determination of the transient concentration of reaction species. Thus, the technique of flash photolysis was born (Porter 1950). Further developments of the method were mainly concentrated on increasing of time resolution (down to few nanoseconds using specially designed coaxial flash lamps), improvement of detection techniques, and an extension of application to reactions in liquid and solid phases.
The advent of the laser in early 1960s revolutionized the method. Nowadays, it allows transient kinetics to be recorded with a time resolution of few femtoseconds (e.g., Huber et al. 2002). The dual pulse method (now is known as pump-probe spectroscopy), initially proposed by Porter, allows this unprecedented resolution because after the splitting of a pico- or femtosecond laser pulse to give the strong (pumping) and weak (probing) beams, the latter can arrive to the sample after precisely defined delay time from few femtoseconds to few nanoseconds. One should bear in mind that the light propagates about 1 mm in air within 3 ps. The optical delay unit provides this variable time. Today, this method of ultrafast flash photolysis spectroscopy is one of the most active experimental approaches in physics, chemistry, and biology. In 1999, Ahmed H. Zewail received the Nobel Prize in Chemistry for his pioneering work in this field.
However, this double pulse method has some disadvantages. Firstly, for each time point one needs the renewal of reaction system, and secondly, the temporal accuracy of kinetics is greatly determined by the stability of the pulses. An obvious benefit is the ability to record a transient spectrum at each time point. Therefore, in parallel with Porter’s first flash photolysis system, a setup with a single flash tube was developed in his lab. Here the probe light was provided by a continuous xenon arc or tungsten lamp, which was passed through a monochromator, and detected with a photomultiplier tube connected to oscilloscope. This configuration is most popular nowadays for investigation of reaction kinetics in the nanosecond and slower time domain and particularly in the field of biophysics, where quite complicated multistep relaxation pathways of molecules (proteins, DNA, etc.) are often observed. The kinetic analysis of such long transients needs very high accuracy of detection. Two applications of such laser flash photolysis system are described as examples.
Flash Photolysis of Bacteriorhodopsin
Bacteriorhodopsin (BR) is a 7-alpha helical, transmembrane protein that acts as a light-driven proton pump. The protein absorbs the visible light (max 570 nm) and transfers the hydrogen ions through a biological membrane. This transport occurs in a multistep manner and is accompanied by changes of absorption spectrum (so-called photocycle of BR). Therefore, time-resolved absorption spectroscopy of BR excited by laser pulse is one of the most common experimental methods of investigation of this protein. In Fig. 1, an example of such traces is depicted in which a 5 ns, 5 mJ, 532 nm laser pulse from the Nd-YAG laser was used and the UV-Vis transient traces measured. Global kinetic analysis of these traces together with other wavelengths in the UV-Vis part of spectrum revealed the eight rate constants, their temperature dependence, and five distinct spectral states of the BR photocycle (Chizhov et al. 1996).
Note that the ultrafast pump-probe experiments detect another three rate constants in the femto- and picosecond time domains (Dobler et al. 1988), thus giving 11 experimentally resolved transitions of the proton transportation by BR.
Flash Photolysis of Caged ATP and Actomyosin Kinetics
Two major motor proteins, actin and myosin are responsible for the muscle contraction. Myosin hydrolyses ATP molecule and converts the energy of hydrolysis to the mechanical force imposed on the actin filaments. The minimal reaction mechanism of the actomyosin reaction contains 15 states and 44 rate constants between them (Geeves et al. 1984). Most of the kinetic investigations of this reaction were performed using rapid mixing technique (stopped-flow, quenched-flow, and relaxation methods). Flash photolysis method can substitute some of these measurements with better economy of sample (Weiss et al. 2000). The 5 ns, up to 20 mJ, 355 nm laser pulses have been used from essentially the same Nd-YAG laser as for BR flash photolysis studies. These pulses photolyzed the inactive precursor of ATP molecule (caged ATP) thus initiating the reaction of free ATP with actomyosin. In Fig. 2, transient light scattering traces indicate actomyosin dissociation, and its subsequent reassociation is shown. Analysis of dissociation (exponential) and association (logistic) kinetics provided important values of the apparent second-order rate constant of the actomyosin dissociation and the catalytic activity of myosin.
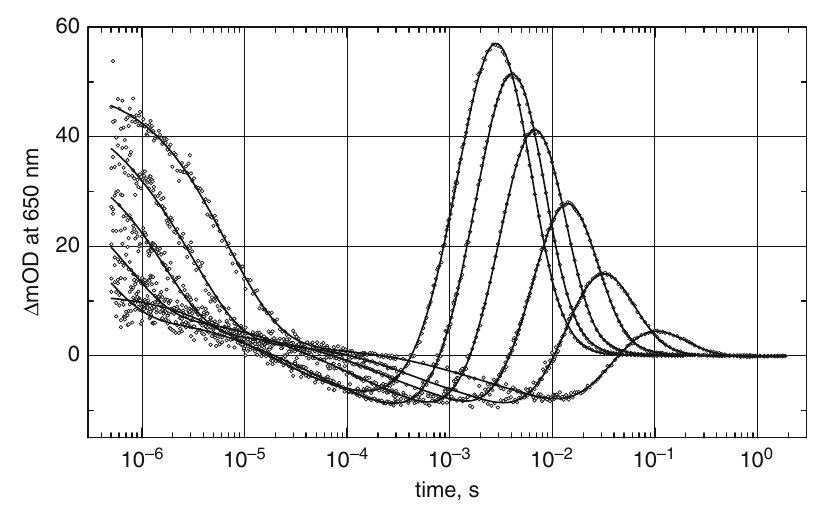
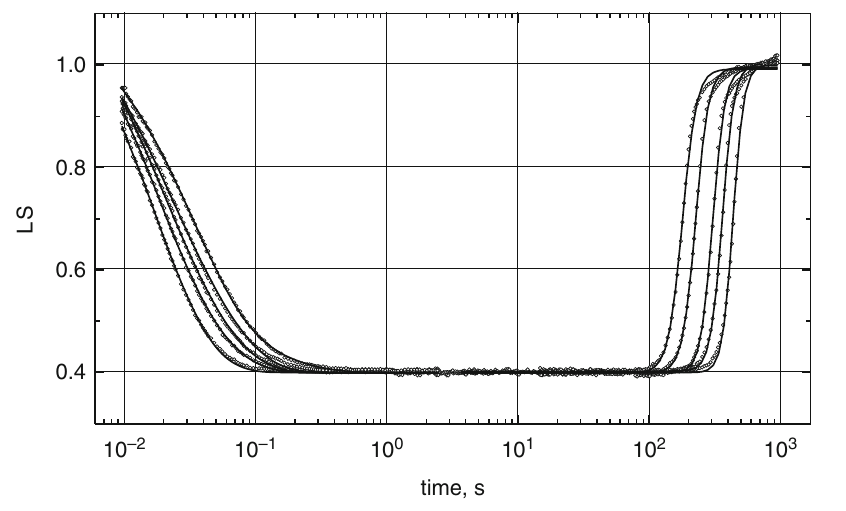
References
Chizhov I, Chernavskii DS, Engelhard M, Müller KH, Zubov BV, Hess B. Spectrally silent transitions in the bacteriorhodopsin photocycle.Biophys J. 1996;71:2329–45.
Dobler J, Zinth W, Kaiser W, Oesterhelt D. Excited-state reaction dynamics of bacteriorhodopsin studied by femtosecond spectroscopy.Chem Phys Lett. 1988;144:215–20.
Geeves MA, Goody RS, Gutfreund H. Kinetics of acto-S1 interaction as a guide to a model for the crossbridge cycle. J Muscle Res Cell Motil. 1984;5(4):351–61.
Huber R, Moser J-E, Graetzel M, Wachtveitl J. Real-time observation of photoinduced adiabatic electron transfer in strongly coupled dye/semiconductor colloidal systems with a 6 fs time constant. J Phys Chem B. 2002;106:6494–9.
IUPAC. IUPAC compendium of chemical terminology, 2nd ed. Oxford: Blackwell Science; 1997.
Porter G. Flash photolysis and spectroscopy – A new method for the study of free radical reactions. Proc R Soc Lond Ser A.1950;200:284–300.
Weiss S, Chizhov I, Geeves MA. A flash photolysis fluorescence/light scattering apparatus for use with sub microgram quantities of muscle proteins. J Muscle Res Cell Motil. 2000;21:423–32.
Ultra-fast and extra-long: a novel laser T-jump apparatus for time-resolved spectroscopy of conformational dynamics of proteins.
I. Chizhov and D.J. Manstein
Institute for Biophysical Chemistry, Hannover Medical School
Development of the Laser T-jump experimental method with two infra-red lasers. One laser generates in the aqueous solution the rise of temperature within 10 nanoseconds and the second laser sustains the high temperature up to 10 seconds or longer. This innovation expands the range of the applicability of method. Relaxation techniques such as a pressure-jump, temperature-jump and flash photolysis are powerful tools for the kinetic study of ligand binding and conformational changes of proteins and nucleic acids. We developed a nanosecond T-jump technique that uses two infra-red (IR) lasers. The first laser pulse of 5 ns duration induces a temperature rise in an aqueous solution of 2 μl volume. A so-called Raman laser with a wavelength of 1.9 μm and ca. 100 mJ energy is used for this initial pulse to achieve a 10°C rise in temperature. The heat from this initial temperature jump starts to dissipate significantly after approximately 10 ms due to the heat transfer to the quartz cover glasses that form most of the reaction chamber surface. To avoid this, we use a second IR laser to keep the temperature constant. A continuous wave (cw) Thulium Fiber laser (TLR-15-1940; IPG Laser GmbH) that generates up to 15 W of power at 1.94 μm wavelength is used for this purpose. This laser alone can heat the sample to 100oC within less than 100 ms. Combining both laser systems and using specially developed computer algorithms for modulation of the Thulium laser’s power, we have achieved the flat temporal profile of following the initial T-jump over nine and more time decades, i.e. from 10 ns to 10 seconds or longer. T-jump profiles were calibrated using a temperature sensitive dye Phenol Red. In Figure 1 the transient absorption of Phenol Red at 560 nm is shown at different T-jump experiments. Note that the vertical axis is scaled to the temperature changes. The observed rise of the Phenol Red signal with a characteristic time of 100 ns matches the rate-limiting step of the temperature-induced deprotonation of the dye. The experimentally obtained temporal profile of the Raman T-jump is depicted as a red line. The blue line shows the T-jump profile when only the Thulium laser is used. In the case of the profile shown, the Thulium laser was switched on for 20 ms with the power set to 15 W followed by a gradual reduction to a final power of about 0.1 W. Power was completely switched off after approximately 9 s. As in the case of the Raman laser the initial rise of temperature can be easily modulated to achieve a higher or lower plateau temperature. Once the plateau temperature has been reached, the time over which the temperature is kept constant can be extended by modifying the computer algorithm that controls the power modulation of the Thulium laser. An example of temperature dependent conformational equilibrium obtained in the Bacteriorhodopsin photocycle illustrates the method in figure 2. The T-jump system described here will be of great use in the fields of Protein Science, Biotechnology, Material Science, Nanotechnology and Chemistry. The temporal overlap between the time domain that can be experimentally observed using our T-jump system and advanced computational approaches that are used to simulate macromolecule dynamics is of particular importance.
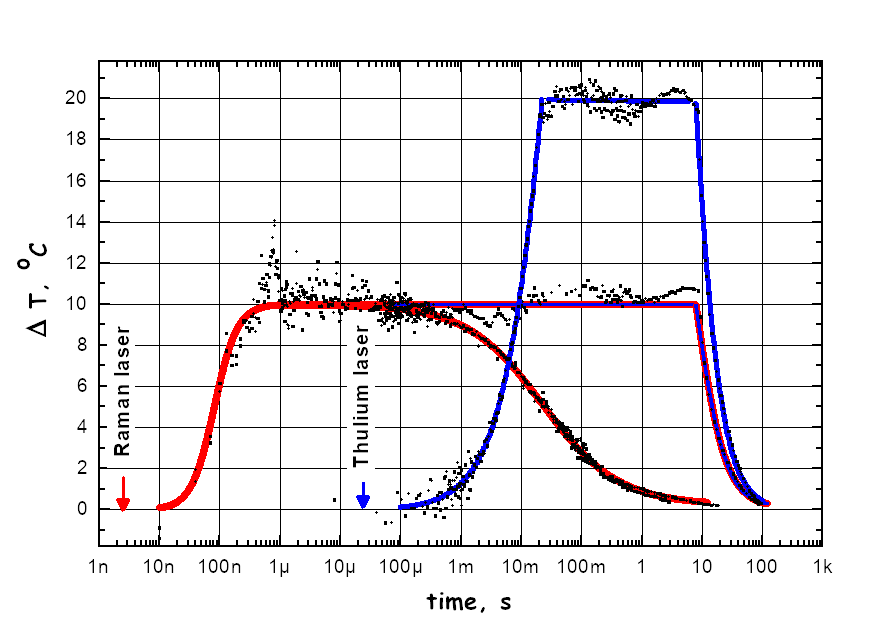
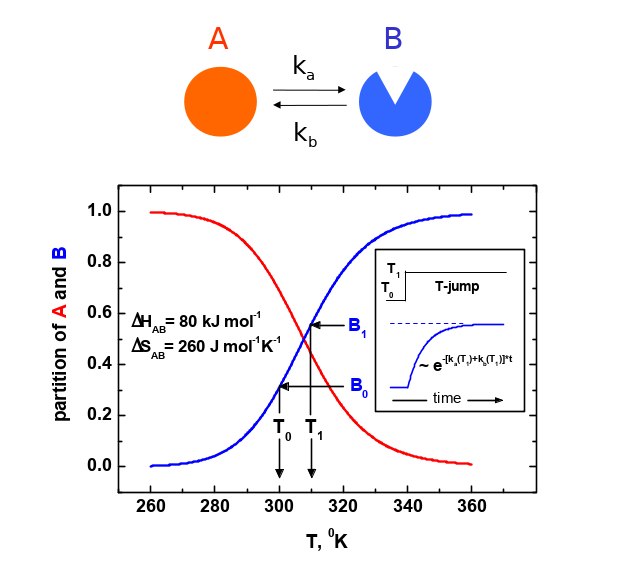